Usability Of Three-dimensional Printing in Maxillofacial Surgery: A Narrative Review
Abstract
Purpose:
The three-dimensional (3D) printing method is a modern approach in which different custom designs are fabricated with high complexity according to the patient’s need. This narrative review aimed to highlight the materials used in 3D printers for medical use, especially in the field of oral and maxillofacial surgery.
Methods:
PubMed, Web of Sciences, and Google Scholar were searched for the relevant studies, and after meeting the inclusion criteria, articles were studied, and focused points were highlighted.
Results:
s: Synthetic and natural materials used in 3D printing include hydroxyapatite, tricalcium phosphate, bicalcium phosphate, apatite–wollastonite glass ceramics, stem cells, and collagen. The most frequent clinical applications include dental implants, maxillofacial trauma, facial cosmetics, orthognathic surgery, maxillofacial oncology, and maxillofacial reconstruction. Anatomical models and surgical instructions were the most often printed objects. The key benefits were increased surgical precision and a shorter operating time. The cost of the items, the length of the manufacturing process when printed by the industry, and legal concerns were the main drawbacks.
Conclusion:
The 3D models are beneficial for surgeons as they can save time and even human life. In the future, additional research should be done on the modeling, efficacy, and safety of natural materials, and systematic reviews and meta-analyses should be conducted for a better understanding.
1. INTRODUCTION
Three-dimensional (3D) technology uses additive manufacturing (AM) techniques instead of the subtractive and formative processes used in conventional production. This process reduces waste material significantly, shortens lead times, and enables the production of complicated geometries that defy conventional engineering standards [1]. The approach which was introduced in the 1980s that became the cause of much attraction among the population highlighted in a report that the compound annual growth rate of the AM industry [2], including all services and products worldwide [3], has grown by 26.2% over the last 27 years to $5.1 billion in 2015 [4].Meanwhile, the healthcare industry is the third largest market globally, with approximately more than 16% overall revenue generation [5]. In today's practice of precision medicine and individualized therapies, patient-specific anatomical models that are 3D-printed are becoming increasingly helpful tools for the medical community [6]. The quality of 3D-printing applications is continually advancing, leading to increased patient usage [7]. Customized, sterilizable, and biocompatible parts are increasingly in demand in the medical industry. The 3D-printing technology offers numerous opportunities for research and development in the medical field [8]. Currently, 3D printing is used in the medical field for research, equipment modification or manufacturing, patient care, and medical education [9]. Also, it is employed in the fabrication of tissue and organs, devices, anatomical replicas, and other medical applications and surgeries such as craniomaxillofacial (CMF) surgery [10].
Currently, 3D printing appears to be more employed in oral and maxillofacial surgery (OMFS), especially since the introduction of 3D printers for general usage a few years ago [11]. Surgeons specializing in OMFS are uniquely positioned to advance and use this technology [12]. Moreover, it allows the surgeon quickly alter the tools and implants to suit certain requirements.
3D printing offers various unique possibilities for creating both generic and patient-specific medical tools [13]. Dental implant surgery and mandibular reconstruction were the two most common clinical indications, and surgical guides and anatomical models were the most commonly printed objects. The prints were professionally done in 45% of the cases. The key benefits were improved precision and a shorter surgical time [11]. Besides the instruments and devices, the pharmacological field is growing, focusing on individualized dose medicines and in different aspects of academia [14]. In addition, 3D-printed medications are revolutionizing the pharmaceutical industry with the development of prospective instruments for achieving personalized treatments tailored to each patient's unique needs, taking into account their age, weight, comorbidities, pharmacogenetic, and pharmacokinetic characteristics [15]. Bio-printing of pre-clinical, patient-specific tissue and disease models for drug testing and high-throughput screening is another emerging area with great potential for developing patient-tailored drugs and reducing the use of animal models [16]. Thus, this review was designed to highlight and analyze available literature regarding the usability of 3D printing in OMFS with the following objectives:
- Different materials are used in the field of medicine for 3D printing.
- Application of 3D printing in different fields of OMFS.
2. METHODOLOGY
Without a time limit, research publications from databases such as PubMed, Web of Sciences, and Google Scholar were searched using the terms “maxillofacial surgery” AND “3D printing” and “maxillofacial surgery” AND “computer-aided design,” respectively. Human clinical usage and English as the publishing language were the inclusion criteria. Articles without online abstracts, those involving micro- or nanoscale 3D printing, animal studies, and studies that were just updates or literature reviews were excluded (Fig. 1).
3. RESULTS
3.1. Different Materials/Biomaterials used in 3D Printing
Materials/biomaterials are synthetic or natural substances used in biological systems to support the replacement, long-term repair, or enhancement of any tissue or organ of the body [17]. These support materials are useful as they can be easily removed at any desired stage with a cutting tool or by hand. However, the observed problem with these materials is the usage of wastage materials from their production and the possibility of leaving an imprint on the surface that further requires polishing treatment to maintain and obtain good-quality printing [18]. For very few cases, it has been observed that model damage loss due to any materialistic factor occurred at any stage, with breakage as the problem and a high device breakage ratio [19].
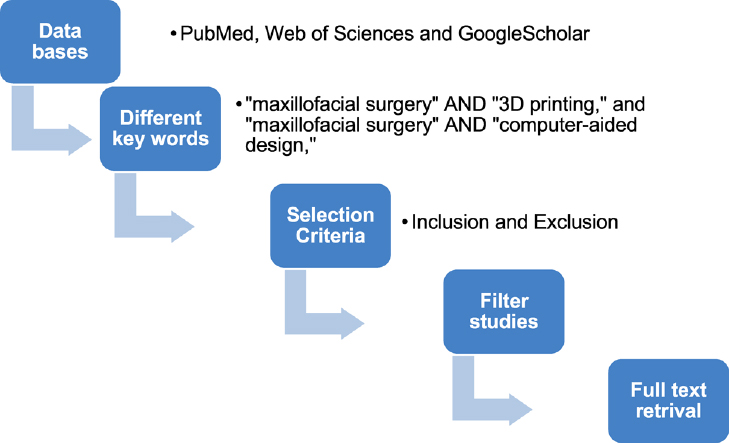
Material Used | Advantages | Disadvantages | Usage | References |
---|---|---|---|---|
Calcium phosphate alone, calcium polyphosphate and polyvinyl alcohol, BCP, TCP, β-tri-calcium phosphate, HA, and TCP | Biocompatible, water-soluble, well extruded, and exhibits strength after hardening up to 4 MPa, improving the mechanical strength | A little amount of ceramic additives compared to photocurable polymers. Due to thermal binding, drugs and/or growth factors cannot be incorporated. | Preparation of medicines, bone regeneration | [22-31] |
Porous ceramic scaffolds (HA) | Extremely osteo-conductive, biocompatible, and non-toxic | Brittleness | Bone tissue | [32, 33] |
SrO and MgO as dopants in TCP | SrO and MgO dopants dramatically increased osteoid, bone, and haversian canal development as compared to pure TCP scaffolds in 3DP TCP | Poor mechanical strength | Tissue regeneration | [34] |
Hydroxyapatite/A–W glass ceramic composite | Bioactive and non-toxic | Low mechanical properties | Bone implant | [35] |
Collagen and calcium phosphate composite | Osteoconductivity and biocompatibility with sufficient mechanical properties | Lack of mechanical strength | Bone graft substitutes | [36] |
Poly-lactic-co-glycolic acid (PLGA) | Adjustable biodegradation and biocompatibility | Low mechanical strength | Bone repair | [25, 26] |
Porous calcium polyphosphate | High compressive strength | The pore orientation of the porous scaffolds significantly impacted their compressive strength and modulus. | Articular cartilage tissue engineering | [22, 37] |
Polycaprolactone (PCL) | High strength | Hydrophobicity and the lack of specific cell recognition sites confined their practical application | Bone tissue repair | [38-40] |
PCL-βTCP | Biocompatible, biodegradable | NA | Alveolar bone defects | [41] |
Aliphatic polyester of organic origin | A heated bed is not required; the material shrinks little, has decent durability, is insoluble, and does not release any toxic fumes. | Greater expenses are associated with processing and production and the potential for deformation brought on by high temperatures. Restricted adaptability |
Regenerative medicine, synthetic organs, and tissues | [42, 43] |
Silk Alginate Chitosan Agarose |
High degradability, biocompatibility, and loading of bioactive molecules | Low mechanical strength | Mandible reconstruction | [44] |
Embryonic stem cell-induced pluripotential stem cell (iPSC) Adult stem cell (ASC) |
Potential to develop into several composite mandibular tissues | Decreased angiogenic capacity and healing | Mandible reconstruction | [44, 45] |
Anti-infective and chemotherapeutic filaments | Ease of placement, reduced hospital stay, decreased systemic toxicity, and less patient cost | Deleterious effects are brought on by thermal damage after polymerization of polymethyl-methacrylate, insufficient biocompatibility, inadequate antibiotic release, and requirement for surgical removal. | Used to make catheters, discs, beads, and other medical devices | [46, 47] |
However, selecting the material that meets the standard requirements is also important for medical device correction and the selection of the printer and process for 3D printing [20]. Similar to other applications, the development of medical applications requires specific mechanical qualities of materials for various anatomical systems to achieve the desired functionality of the object printed [6]. The major difference in the materials can be analyzed based on the different materials in the human body. Soft and hard materials such as “human bones” are the best examples of hard tissue, and “ligaments or articular cartilage” are the best examples of soft materials. It has been proven in different studies that in 3D printing, bones are the most straightforward and uncomplicated biological tissue to produce because most of the elements are hard [21]. The ideal biomaterial for 3D printing should mirror the morphology of live tissue, have customizable degradation rates, and be easily printable [17]. Materials/biomaterials used for 3D printing are stated in Table 1.
3.2. 3D Printing in the Field of OMFS
3D printing in OMFS first gained popularity in the 1990s, when anatomical bio-models were printed to help with surgical planning for patients with severe craniomaxillofacial abnormalities [22-48]. Since then, the application of bio-models has expanded to include complex maxillofacial trauma, head and neck oncology, orthognathic surgery, and other areas of OMFS [49]. It can model hard and soft tissues and colorize pathology or other interesting structures. In addition, it guides practitioners in preoperative treatment planning. It is simple to virtually design and perform surgical procedures with rapid prosthesis loading [50]. OMFS has made ground-breaking breakthroughs by advancing 3D printing technologies [51], which can be divided into four categories: implants, splints, contour models, and guidance. The diverse objectives of maxillofacial and craniofacial surgery are reflected in the utilization of these four techniques. This knowledge could aid in developing and forecasting the application of 3D printing for further plastic surgery procedures [52]. Currently, tissue engineering, difficult temporomandibular joint restoration, trauma surgery, pathology-induced abnormalities, and sophisticated facial asymmetry correction are all current uses of 3D printing in OMFS [53] and other applications as it improves precision, predictability, and accuracy while cutting costs and time, hence improving patient outcomes [54].
The use of such technologies in surgery can primarily be divided into three main areas:
3.2.1. Preoperative Planning
Clinical staff can more easily understand complex anatomical information with the aid of 3D-printed models, which is helpful for pre-surgery planning, surgical training, and intraoperative navigation [55]. The anatomical model fabrication based on patient data is made possible by the application and development of advanced technologies in the field of 3D printing, such as AM and rapid prototyping in concurrence with medical imaging techniques [56]. Medical anatomical models have a broader range of use as tools for surgical planning owing to the incorporation of technology for the rapid fabrication of digital pictures [57]. They enable both virtual and physical preoperative simulation using a 3D-printed model. By using 3D fast printing technology, the data provided by cone beam computed tomography (CBCT) enables the creation of these 3D models.
3.2.2. Virtual Printing
Pre-contoured plates and grafts are virtually planned and printed to enhance surgical outcomes and shorten recovery times. Reconstruction accuracy and operating time for mandibular microvascular surgeries can be improved with preoperative planning and printing using rapid prototype modeling and computer-assisted design [58].
3.2.3. Practice implications and simulation
Models for education and training offer the chance to practice surgical techniques, better visualize anomalies, forecast potential issues, and enhance quality by lowering errors [59]. Thus, utilizing the model might result in lower training costs and more patient security [60].
3.3. Dental Implants
Dental implants are a widely used popular treatment option for tooth loss. They are essential for treating various dental issues, such as tooth loss, crown damage, and diastema [61]. Computer-aided design was introduced in dentistry in the 1970s [62]. Although 3D presentation has long been introduced in the dental field, some procedures are still performed manually, with error risks in all types of tooth manufacturing (Impellizzeri et al., 2020). Thus, as a substitute method, 3D printing or AM with metal and plastic was used to create the prototype abutment, computer-aided design, scanning, and 3D printing of plastics and metals [63]. Meanwhile, alveolar bone resorption is the main dentary element that improved over time in 3D representation, although it has poor retention and stability of previous treatment. Different implants are available for dental treatment; however, 3D printing is the best representation of the human body utilized due to some features such as length, representation, and attraction [64]. Nowadays, the modern dentistry trend is moving towards two main directions; photo polymerization and powder-based printing [65]. Their advantages include rapid fabrication, high resolution of dental procedures, cost-effectiveness, and flexibility to adapt to the structure of the shapes and in-depth surfaces of the body [66]. In dental surgery, validity and accuracy representation with no harm or zero risk element is essential. A study assessed the complication and accuracy of selective-laser sintering surgical guides for tooth implantation and placement [67]. In the study, angular and lateral deviations between the implants and virtual considerations were performed in 60 dental implants, and results were evaluated during the proper study follow-up. The study can be considered a long-term study as the follow-up period after surgery or impanation was 30 months. The results indicated that both the complication rate (34.4%) and apical deviations (>2 mm) were high instead of the presentation of the manufacturing element [68].
In addition, the accuracy of the implementation of tooth surgery has been guided by following the conventional techniques with additional manufacturing of the stereolithography (SLA) & multi-jet modeling (MJM) fabrications [69]. However, regarding the fabrication accuracy with SLA, conventional fabrications are more accurate than other guidelines in dental surgeries [70]. Other than the surgery, different studies have indicated the filling of gaps with direct metal laser sintering prostheses, which represents slightly higher accuracy; however, this warrants further investigation.
3.4. Maxillofacial Trauma
Several mild to life-threatening injuries can be present in patients diagnosed with maxillofacial trauma. Intermaxillary fixation is no longer the standard of care, as complex fractures can result in functional and cosmetic impairment [71]. Although 3D printing is widely employed in CMF surgery, the use of this technology is restricted in cases of severe trauma because outsourcing takes too long [72]. However, 3D-printed life-size models are becoming a more helpful adjunct during surgery for comminuted face fractures. They aid plate bending and preoperative surgical practice, enabling anatomical reduction with less operative time and cost [73].
The treatment of trauma patients with delayed or recent fractures and deformities is made easier by 3D printers [74]. Although 3D printing technology is typically employed in craniofacial surgery, some studies have demonstrated that it can also be used to treat nasoethmoid orbital fractures [75]. Treatment options for these patients include titanium mesh or sheet-based 3D restoration of the orbital wall deformities [76]. Compared to the manual-bending implant, the 3D-printed standardized implant offers surgical efficacy in repairing inferomedial orbital fractures [77]. Additionally, the 3D printing methodology proved relevant in acute midface trauma and produced positive results. Implementing 3D printing in the present care of acute midface trauma requires a thorough grasp of the procedures required to create the stereo lithic model [78]. Also, a crucial nonverbal method of communication, facial expression, may be impaired by motor nerve and soft tissue injuries from scarring [79]. Changes to occlusion and speech may also result from non-anatomical repairs to the related soft tissues and underlying facial bones. Without considering dental rehabilitation, oral cavity reconstruction will make prosthetic results more difficult to achieve after healing [79]. Moreover, by using a specially developed poly-DL-lactide implant in conjunction with virtual preoperative modeling, blowout fractures of the orbital floor can be repaired with satisfactory functional and aesthetic results [80]. In a case study, a male patient with a comminuted facial and skull bone fracture was given a 3D-printed, specially designed-polymethyl methacrylate prosthesis to restore facial bone and frontal bone abnormalities. Excellent patient, family, and surgeon satisfaction were evident after a 10-month postoperative follow-up for a fraction of the price of commercially available implants [81]. A meta-analysis used indicators like operation time, blood loss during the procedure, the number of fluoroscopies performed during the procedure, the time it took for the fracture to heal, and the percentage of patients with outstanding outcomes to demonstrate that surgery with the help of 3D printing is a better option than traditional surgery for treating traumatic fractures. Furthermore, the meta-analysis revealed no discernible variations in the occurrence of complications between the two treatment methods [82].
3.5. Facial Cosmetics
Compared to conventional manufacturing methods, 3D printing has some benefits, including one-step fabrication and customization [83]. Additionally, 3D printing can potentially improve user compliance and the effectiveness of skin delivery [84]. Broadly, two types of 3D-printer-made materials can be used as facial cosmetics (microneedles (MNs) and skin patches).
The study of cosmetic MNs manufactured via 3D printing is in its early stage. However, numerous articles and patents have demonstrated the viability of delivering both lipophilic and hydrophilic active substances using MNs that are made using techniques other than 3D printing [85, 86]. Furthermore, the emergence of a painless controlled-release drug delivery system has been made possible by the invention of 3D-printed MNs [87]. After 8 weeks of therapy, a study assessed the effectiveness of dissolving MNs into two standard formulations (both of which contain hyaluronic acid) in reducing wrinkles [88]. The MNs were shown to be more effective. All MNs improve skin delivery through the micro-channels they build, partially avoiding the skin barrier. There is a second mechanism for wrinkle improvement due to the perforations they produce; elastin and collagen may be expressed and deposited, promoting metabolism in the higher skin layers and skin healing on its own [89, 90]. The material that 3D-printed MNs are made of largely determines their effectiveness. A wide range of materials, including carboxymethylcellulose-based polymers [91], polyester resin, polyvinyl alcohol/polylactic acid, and acrylonitrile butadiene styrene, have been researched for this purpose [92].
Another method used for facial cosmetics is skin patches made of 3D printers, and these microarray patches (MAPs) comprise several microscopic projections that can easily pierce the skin to access the epidermal/dermal layer without causing pain [93]. These patches also have exceptional bioactivity to speed wound healing and superb photodynamic therapy-based anti-infection performance [94]. MAPs have been widely used to quickly deliver medicines to the skin in aesthetic contexts. The derma roller, a tool with numerous 0.5–1.5 mm length needles organized on a roller device, is one of cosmetics' most well-known MAP applications. By piercing the stratum corneum to create micro conduits that stimulate growth factor secretion and collagen formation, derma rollers have been used to treat stretch marks and acne scars [95].
In a previous study, SLA printing produced 3D-printed devices (nose-shaped) with higher drug loading (1.9% w/w) and resolution than fused deposition modeling [96]. The findings of the drug diffusion experiments showed that, for the two formulations tested, drug diffusion was 229 and 291 g/cm2 faster than with the fused deposition modeling devices within 3 hours. In contrast, SLA printing was discovered to be the most suitable technology for 3D printing for producing salicylic acid-based anti-acne devices [96]. Additionally, a study reported that nano-cellulose devices did not allow bacterial growth when the nano-cellulose bio-ink was used to print 3D porous structures, which is an intriguing characteristic of these novel materials [97].
3.6. Orthognathic Surgery
Computer-assisted surgical simulation and planning are now frequently employed for examining craniofacial anatomy and enhanced surgical success prediction in orthognathic surgery due to recent advancements in 3D imaging [98]. Different types of orthognathic surgery include osteotomy guide, occlusal splint, fixation plate/implants, and spacer [98].
3.6.1. Osteotomy Guides
For the repositioning guide to precisely position the bone segment in its position correctly, osteotomy guides are utilized to ensure that the osteotomy is performed exactly as in the digital planning. 3D-printed osteotomy guides have been documented for bilateral sagittal spilt osteotomy (BSSO) and genioplasty surgery. Numerous studies have emphasized the use of surgical guides for condylar position management and inferior alveolar nerve injury avoidance [99, 100]. In addition, the placements of the screws and osteotomy lines were transferred to the operation room using a surgical guide that was made. The method is quick and simple to use, and the postoperative results are encouraging [75].
3.6.2. Occlusal Splints
Occlusal splints are frequently employed in dentistry practices for various reasons. They are utilized as an extra treatment method for temporomandibular disorders to either loosen the muscles or permit the condyle to sit in centric relation and protect the teeth and associated structures during bruxism [101]. The first full-coverage and flat-plane occlusal splints with guidance ramps to be designed and manufactured using computers were proposed by Lauren in 2008 [102]. In a prospective trial, researchers investigated the effectiveness of rapid prototyping-created combination guiding templates and splints for treating facial asymmetry caused by vertical maxillary excess and mandibular prognathism. They observed that this method could be more accurate, complex, and time-efficient than conventional methods [103].
3.6.3. Fixation Plate/Implants
The manufacture of titanium mini plates/guides using direct metal laser sintering technology was encouraged by Philippe [104]. Other studies also reported employing patient-specific titanium plates made with the electro-optical systems (EOS) titanium Ti64 technology for repositioning and fixation of the maxilla segment without requiring surgical splints. There is a pre-alloyed Ti6AIV4 alloy that has outstanding mechanical and corrosion-resistance characteristics, a low specific weight, and is extremely biocompatible. A biomedical implant can easily be made with the material [100, 105]. Also, an ideal biomechanical miniplate was created, and the plates achieved accurate positioning/fixation and demonstrated acceptable strength in the LeFort I osteotomy when attached to the liberated separate maxillary segments of a fast prototyping model [106].
3.6.4. Spacers
Spaces are created in the LeFort I procedure when the maxilla vertical dimension is lengthened, in BSSO after the mandible is rotated or shifted to maintain or increase the space in cheek contour correction or symmetry, and in genioplasty when the vertical dimension of the maxilla is lengthened. For the Le Fort I and mandibular ramus segments, a study evaluated a total of 19 spacers in 12 patients. The spacers performed admirably during the bone-fixing procedure. The average visual analog scale scores before and after surgery were 4.83 and 7.14, respectively, with statistically significant improvement in face symmetry (p = 0.018) [107].
4. MAXILLOFACIAL ONCOLOGY
Head and neck tumor resections and reconstructions have been transformed by 3D printing. A new era of “digitalization and precision surgery” has emerged from cutting-edge technology in head and neck reconstruction, using 3D printing and virtual surgical planning of surgical guides and implants customized for each patient [108]. A 3D-printed tumor aids reliable investigations on metastasis. These models establish an encouraging framework for building biomimetic models. Furthermore, this technology appears to be the best instrument for facilitating surgery, complex treatment, and therapies because it allows accurate in vitro model fabrication [109]. Additionally, it can be used in clinical practice to lessen the discomfort associated with cancer therapy. It might be compared to employing chemotherapy and radiation therapy as long-lasting cancer treatments [110]. Tumor cells are extracted and printed during this process. This makes it easier to test various medications and choose the best course of action for the patient [111]. In a case study, a patient with mucoepidermoid cancer of the maxilla removed surgically had rapid repair with a vascularized free fibula flap made with virtual surgical planning. The midface and maxilla were reconstructed to optimum dimensions using a customized 3D plate printed from titanium that precisely matched the surgical defect, requiring no unforeseen surgical intervention and less operative time [112]. In addition, spheroids, scaffold-based, and organoid constructs are examples of 3D in vitro models that can reproduce the three dimensions of tumors and help us better understand the role that different micro-environmental cues play in the development and spread of oral cancer. However, because these conventional tissue engineering techniques cannot manage how various cell types are organized in a complex architecture, they cannot properly replicate the heterogeneity of the tumor microenvironment [113].
5. MAXILLOFACIAL RECONSTRUCTION
Deformity reconstruction is among the most complicated techniques in head and neck surgery. The “gold standard” of reconstructive and regenerative techniques for OMF malformations is still the transfer of various auto-grafts [114]. However, harvesting these grafts can result in complications, such as lengthening of the surgical procedure, donor-site morbidity, insufficient donor-site healing, etc [114]. The basic objectives of craniofacial reconstruction are the restoration of intricate anatomical, functional, and cosmetic features, with special consideration for the craniofacial development of growing patients. Due to their osteoconductive and osteoinductive properties, osteogenic properties, and the potential for the continuous growth of specific autologous grafts at the sites of defect that is the costochondral graft, autologous bone grafts remain the gold standard in hard-tissue reconstructive surgery [115, 116].
Facial reconstruction surgeries benefit from 3D printing. Before the procedure, the implant is customized on a 3D surgical model to minimize tissue stress and operating time [117]. Although the development of bone tissue engineering techniques (3D printing) has completely changed the area of maxillofacial reconstruction, a substantial obstacle remains regarding the successful translation of such products, particularly for larger-sized defects [118]. This method can enhance both the aesthetic result and functional rehabilitation when utilized in jaw repair [119]. The use of 3D printing in jaw repair has been documented in numerous studies; however, little is acknowledged about its clinical advantages over traditional surgical methods. In addition, the majority of the data are derived from case series and reports that only include a few patients and lack comparators [120]. Advances in customized medicine have been significantly impacted by 3D printing, with craniofacial reconstruction leading many of the main discoveries over the past 10 years [121]. This technology has advanced with the development of tissue-engineered bone grafts, which might allow for more widespread application. They offer a promising substitute that enables precise graft shaping, eliminating the need for multiple operations and the resulting comorbidities [122]. In prospective research, individuals with mandibular or maxillary abnormalities with printed titanium mesh reconstruction utilizing computer-assisted surgery (CAS) were examined. All patients received an excellent contour. For the mandibular and maxillary reconstructions, respectively, there was <81% and 94% within 3 mm rate of concordance between the postoperative outcome and preoperative design [123].
Generally, there are two types of implants used for CMF reconstruction; synthetic and biological. Synthetically manufactured scaffolds may not exactly duplicate the structural or biochemical characteristics of native scaffolds; however, they provide more control over the production process and have more dependable repeatability [124]. For particular purposes, synthetic grafts can have their material characteristics, bioactivity, porosity, and form precisely regulated. PCL and polyetherketoneketone are two recent compounds that have emerged as useful polymers for scaffold building. The PCL has demonstrated biocompatibility; breakdown occurs safely in the body at a pace comparable to the creation of new bone, and it has already been given regulatory approval for some uses [125, 126].
Alternatively, biological scaffolds offer higher osteoconductive qualities, better implant integration, and the possibility of lengthening lifespan. Numerous studies have shown that a variety of printed substrates enable the formation of functional bone tissue and vasculature by human adipose-derived stem cells compared to smooth two-dimensional (2D) scaffold controls, and human bone marrow stromal cells cultured on 3D scaffolds show higher proliferation and growth. Moreover, 3D scaffolds can bind more protein because they have a bigger surface area than smooth 2D scaffolds [127]. Bypassing the size limitations and resultant comorbidities of conventional bone graft harvesting is a crucial step in demonstrating the capability and promise of these grafts. With the help of this technology, precise anatomical patient-specific implants could become more durable and accurate as the body continues to change and integrate them.
6. DISCUSSION
Clinical outcomes have significantly improved as a result of the application of 3D technology for medical interventions, ranging from straightforward surgical operations to fracture reduction and defect correction [128, 129]. The unaffected side is mirrored, then models are printed, commercial plates or meshes are pre-bent, or patient-specific instrument design is used. These techniques have improved accuracy and demonstrated speed [130]. The initial phases in the 3D printing process involve the processing, safe transfer, and digital reconstruction of anatomic scans. Due to its greater spatial resolution and hard tissue contrast, CBCT often takes the form of a fine-slice computed tomogram; however, magnetic resonance imaging is also beneficial for virtual surgical planning in circumstances when ionizing radiation may be contraindicated [131].
In this study, the materials used in 3D printers for medical use and the application of 3D in the area of OMFS were reported. The different materials used, synthetic and natural, are listed in Table 1. Selecting the material that meets the requirement of the model is also important for fabricating a suitable medical device and selecting the printer and 3D-printing process [20]. To achieve the desired performance of the printed product, different anatomical structures require varying mechanical qualities of the materials, just like in other applications. Both types of materials can be used; however, they have their strengths and limitations and should be used according to the need or purpose.
Synthetic materials, mostly polymers and ceramics, are used in medicine, especially for bone regeneration and implantation, with outstanding customizable chemical structures, mechanical qualities, non-toxic degradation products, and predictable breakdown rates, making them ideal candidates for producing models [132]. Conversely, natural materials such as silk, embryonic stem cell-induced pluripotential stem cells, adult stem cells, chitosan, alginate, and agarose are the few examples mentioned in this study. The main advantages of natural materials for 3D printing are plentiful and diverse with limited side effects. They can be employed as a natural material with cell adhesion, stability, and biocompatibility to increase usage and obtain desired results. Further, with the addition of some stabilizers, they can be used to create composite scaffolds or composites for 3D bio-printing, which are becoming increasingly crucial in various fields, including regenerative medicine and tissue engineering [133].
In this narrative review, the main indications of the 3D printer in the field of OMFS were presented, and the main focus was on dental implants, maxillofacial trauma, facial cosmetics, orthognathic surgery, maxillofacial oncology, and maxillofacial reconstruction. Surgical guides are the most commonly produced 3D items for dental implant surgery and are intended to make drilling and orienting easier to aid accurate implant placement, as expected in preoperative planning [134]. In addition, surgical guides are the most often printed 3D items in mandibular reconstruction. These tools are designed to aid the surgeon in obtaining the proper angulation and positioning of the osteotomy lines, placing the screws in predetermined locations on the model, and positioning the osteotomized bone segments in accordance with engineering and regenerative medicine planning. It was concluded that these guides play a very important role in dentistry; however, the main concern is their registration and approval from the regulatory authorities. The use of 3D-printed models in maxillofacial trauma cases has been reported; however, the models may cause deformities with long-lasting social and psychological effects on patients. Nonetheless, the application of 3D printing is very useful in these cases, and different 3D meshes can be used to regenerate skins and implants for the facial bone. According to the literature, it has a significant effect on the improvement compared to conventional techniques, and the fabrication process is easy; however, the only problem is the legislative problems as the use of commercially available “biocompatible” and/or “sterilizable” filaments which are used to print sterilizable surgical models is currently prohibited under Canadian and American standards. Thus, internal models may be used to pre-bend sterilized implanted parts prior to surgery, or the models may need to be encased in plastic bags (sterile) if used in the operating room [72].
In facial cosmetics, two types of 3D models are used. They include MNs and patches, which have very good results. These techniques are used for acne and wrinkles removal, and patches can also be used for topical medicine delivery. In addition, dissolving MNs have the greatest potential among them, and 3D-printed MNs would replace patches for both medical and cosmetic uses. The application of 3DP technology seems to be a promising strategy for creating effective platforms for delivering customized cosmetics [135].
Other focused fields are orthognathic surgery, maxillofacial oncology, and maxillofacial reconstruction. Research has highlighted the need to meet the client’s requirements and outcome satisfaction level. Different types of orthognathic surgery were identified in this study, including osteotomy guide, occlusal splint, fixation plate/implants, and spacer. Meanwhile, high biomechanical criteria are not necessary for the 3D-printed anatomical models, surgical guides, and occlusal splints that have been published in the literature. They only utilize readily available hard and software technologies and simple materials such as resins, plastics, resorbable polymers, etc. This likely explains their widespread use, which is sometimes wholly prohibited; materials should be used according to the country's regulations.
In maxillofacial oncology, 3D models assist surgeons in planning and studying tumor models. Similarly, the restoration of complex anatomical, functional, and aesthetic aspects is the main goal of craniofacial reconstruction, with specific attention paid to the craniofacial growth of growing patients. Due to their osteoconductive, osteoinductive, and osteogenic qualities and the possibility of some autologous grafts continuing to grow at the sites of defects, autologous bone grafts remain the gold standard in hard-tissue reconstructive surgery (i.e., costochondral graft).
Overall, this review revealed the importance of 3D application in the field of OMFS; however, there are research gaps in the safety and effectiveness of the materials used for 3D printers. Therefore, a systematic review and meta-analysis should be planned for a better understanding and outcome when compared with the conventional method.
CONCLUSION
In this review, the understanding of 3D printing introduction and its impact on the medical field has been extensively highlighted with the exploration of the studies in the field of OMFS. This review emphasized that 3D printing is widely used and accepted as a manufacturing technique with less time in addition to its huge impact on the specification of specialization, cost-effectiveness, and on-demand fabrication. Based on the literature searched, different synthetic and natural materials used for 3D printing have been highlighted. 3D application in the field of OMFS, especially in dental implants, maxillofacial trauma, facial cosmetics, orthognathic surgery, maxillofacial oncology, and maxillofacial reconstruction, was also highlighted. The quality and cost-effectiveness of 3D printing while maintaining the safety risk behaviors according to the severity of the issue and legislative issues were also considered. The materials used for implants and guides for modeling should be registered, and the byproducts should be free of infections since they are used directly by humans. Further research should be conducted on the effectiveness and safety of natural materials, and a systematic review and meta-analysis should be conducted for a better understanding.
LIST OF ABBREVIATIONS
HA | = Hydroxyapatite |
TCP | = Tricalcium Phosphate |
BCP | = Bicalcium Phosphate |
RE | = Radix Entomolaris |
A-W glass ceramics | = Apatite–Wollastonite |
3DP | = Three-dimensional Printing |
AVAILABILITY OF DATA AND MATERIALS
The authors confirm that the data supporting the findings of this study are available within the article.
ACKNOWLEDGEMENTS
Declared none.